Vector-Borne Diseases
Key Findings
Key Finding 1: Changing Distributions of Vectors and Vector-Borne Diseases
Key Finding 2: Earlier Tick Activity and Northward Range Expansion
Ticks capable of carrying the
Key Finding 3: Changing Mosquito-Borne Disease Dynamics
Rising temperatures, changing precipitation patterns, and a higher frequency of some extreme
Key Finding 4: Emergence of New Vector-Borne Pathogens
Vector-borne pathogens are expected to emerge or reemerge due to the interactions of
Vector-borne diseases are illnesses that are transmitted by vectors, which include mosquitoes, ticks, and fleas. These vectors can carry infective pathogens such as viruses,
Table 5.1: Summary of Reported Case Counts of Notifiable Vector-Borne Diseases in the United States.
Diseases | 2013 Reported Cases | Median (range) 2004–2013b |
---|---|---|
Tick-Borne | ||
|
36,307 | 30,495 (19,804–38,468) |
Spotted Fever Rickettsia | 3,359 | 2,255 (1,713–4,470) |
Anaplasmosis/Ehrlichiosis | 4,551 | 2,187 (875–4,551) |
Babesiosisb | 1,792 | 1,128 (940–1,792) |
Tularemia | 203 | 136 (93–203) |
Powassan | 15 | 7 (1–16) |
Mosquito-Borne | ||
|
2,469 | 1,913 (712–5,673) |
Malariac | 1,594 | 1,484 (1,255–1,773) |
Dengueb,c | 843 | 624 (254–843) |
California serogroup viruses | 112 | 78 (55–137) |
Eastern equine encephalitis | 8 | 7 (4–21) |
St. Louis encephalitis | 1 | 10 (1–13) |
Flea-Borne | ||
Plague | 4 | 4 (2–17) |
a State
b Babesiosis and dengue were added to the list of nationally notifiable diseases in 2011 and 2009, respectively. Median and range values encompass cases reported from 2011 to 2013 for babesiosis and from 2010 to 2013 for dengue.
c Primarily acquired outside of the United States and based on travel-related exposures.
Vectors and hosts involved in the transmission of these infective pathogens are sensitive to
The seasonality, distribution, and
Climate change is likely to have both short- and long-term effects on vector-borne disease transmission and infection patterns, affecting both seasonal risk and broad geographic changes in disease occurrence over decades. However, models for predicting the effects of climate change on vector-borne diseases are subject to a high degree of
The risk of introducing exotic pathogens and vectors not currently present in the United States, while likely to occur, is similarly difficult to project quantitatively.4,5,6 In recent years, several important vector-borne pathogens have been introduced or reintroduced into the United States. These include West Nile virus, dengue virus, and chikungunya virus. In the case of the 2009 dengue outbreak in southern Florida, climate change was not responsible for the reintroduction of the virus in this area, which arrived via infected travelers from disease-
This chapter presents case studies of Lyme disease and West Nile virus infection in relation to weather and climate. Although ticks and mosquitoes transmit multiple infectious pathogens to humans in the United States, Lyme disease and West Nile virus infection are the most commonly reported tick-borne and mosquito-borne diseases in this country (Table 5.1). In addition, a substantial number of studies have been conducted to elucidate the role of climate in the transmission of these infectious pathogens. These broad findings, together with the areas of uncertainty from these case studies, are generalizable to other vector-borne diseases.1
Figure 5.1: Climate Change and Health—Lyme Disease
State of the Science
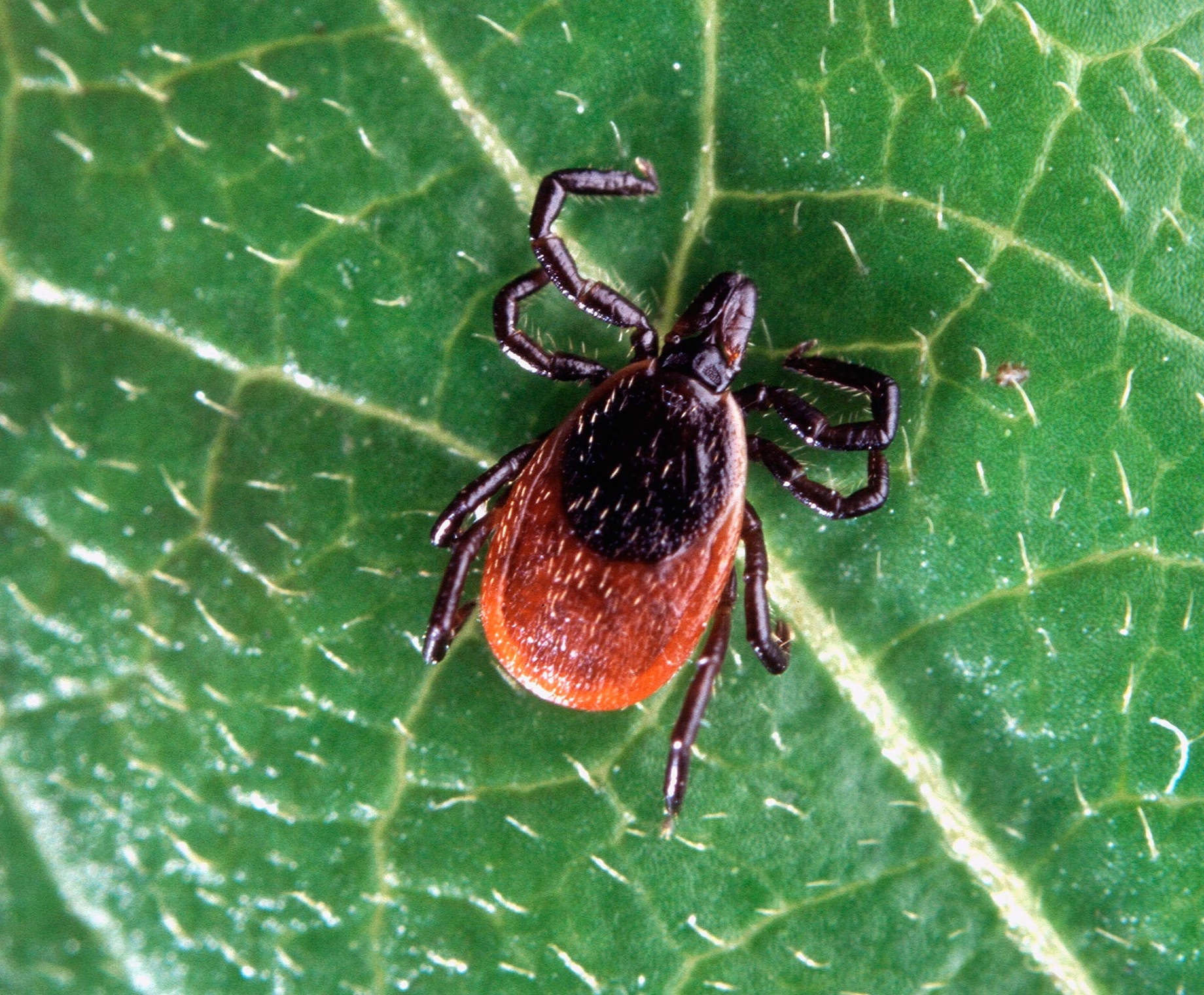
In the eastern United States,
© Science Stills/ARS/Visuals Unlimited/Corbis
Lyme disease is a tick-borne bacterial disease that is
Although the reported
Infection rates in humans vary significantly from year to year. From 1992 to 2006, variation in case counts of Lyme disease was as high as 57% from one year to the next.21 Likewise, the precise week of onset of Lyme disease cases across states in the eastern United States, where Lyme disease is endemic, differed by as much as 10 weeks from 1992 to 2007. Much of this variation in timing of disease onset can be explained by geographic region (cases occurred earlier in warmer states in the mid-Atlantic region compared with cooler states in the North); however, the annual variation of disease onset within regions was notable and linked to winter and spring climate variability (see “Annual and Seasonal Variation in Lyme Disease” below).23
Figure 5.2: Changes in Lyme Disease Case Report Distribution
The geographic and seasonal distributions of Lyme disease case occurrence are driven, in part, by the life cycle of vector ticks (Figure 5.3). Humans are only exposed to Lyme disease spirochetes (B. burgdorferi) in locations where both the vector tick populations and the infection-causing spirochetes are present.26 Within these locations, the potential for contracting Lyme disease depends on three key factors: 1) tick vector abundance (the density of host-seeking nymphs being particularly important), 2)
Aside from short periods of time when they are feeding on hosts (less than three weeks of their two- to three-year life cycle), ticks spend most of their lives off of hosts in various natural landscapes (such as woodlands or grasslands) where
In summary, weather-related variables can determine geographic distributions of ticks and seasonal activity patterns. However, the importance of these weather variables in Lyme disease transmission to humans compared with other important predictors is likely scale-dependent. In general, across the entire country, climate-related variables often play a significant role in determining the occurrence of tick vectors and Lyme disease incidence in the United States (for example, Lyme disease vectors are absent in the arid Intermountain West where climate conditions are not suitable for tick survival). However, within areas where conditions are suitable for tick survival, other variables (for example, landscape and the relative proportions within a community of zoonotic hosts that carry or do not carry Lyme disease-causing bacteria) are more important for determining tick abundance, infection rates in ticks, and ultimately human infection rates.38,44,45
Observed Trends and Measures of Human Risk
Geographic Distribution of Ticks
Because the presence of tick vectors is required for B. burgdorferi transmission to humans, information on where vector tick species live provides basic information on where Lyme disease risk occurs. Minimum temperature appears to be a key variable in defining the geographic distribution of blacklegged ticks.38,44,46 Low minimum temperatures in winter may lead to environmental conditions that are unsuitable for tick population survival. The probability of a given geographic area being suitable for tick populations increases as minimum temperature rises.44 In the case of the observed northward range expansion of blacklegged ticks into Canada, higher temperatures appear to be a key factor affecting where, and how fast, ticks are colonizing new localities.47,48,49,50,51
Maximum temperatures also significantly affect where blacklegged ticks live.38,44 Higher temperatures increase tick development and hatching rates, but reduce tick survival and egg-laying (reproduction) success.29
Declines in rainfall amount and humidity are also important in limiting the geographic distribution of blacklegged ticks. Ticks are more likely to reside in moister areas because increased humidity can increase tick survival.34,37,38,44,46,48
Geographic Distribution of Infected Ticks
Climate variables have been shown to be strong predictors of geographic locations in which blacklegged ticks reside, but less important for determining how many nymphs live in a given area or what proportion of those ticks is infected.38,39 The presence of uninfected nymphs and infected nymphs can vary widely over small geographic areas experiencing similar temperature and humidity conditions, which supports the hypothesis that factors other than weather play a significant role in determining nymph survival and infection rates.36,38,39,40,43 Additional studies that modeled nymphal density within small portions of the blacklegged tick range (north-central states and Hudson River Valley, NY), and modeling studies that include climate and other non-biological variables indicate only a weak relationship to nymphal density.52,53 Nonetheless, climate variables can be used to model nymphal density in some instances. For example, in a single county in northern coastal California with strong climate gradients, warmer areas with less variation between maximum and minimum monthly water vapor in the air were characteristic of areas with elevated concentrations of infected nymphs.40 However, it is likely that differences in animal host community structure, which vary with climatic conditions (for example, relative abundances of hosts that carry or do not carry Lyme disease-causing bacteria), influenced the concentration of infected nymphs.36,54
Geographic Distribution of Lyme Disease
Though there are links between climate and tick distribution, studies that look for links between weather and geographical differences in human infection rates do not show a clear or consistent link between temperature and Lyme disease incidence.45,55,56
Annual and Seasonal Variation in Lyme Disease
Temperature and precipitation both influence the host-seeking activity of ticks, which may result in year-to-year variation in the number of new Lyme disease cases and the timing of the season in which Lyme disease infections occur. However, identified associations between precipitation and Lyme disease incidence, or temperature and Lyme disease incidence, are limited or weak.57,58 Overall, the association between summer moisture and Lyme disease infection rates in humans remains inconsistent across studies.
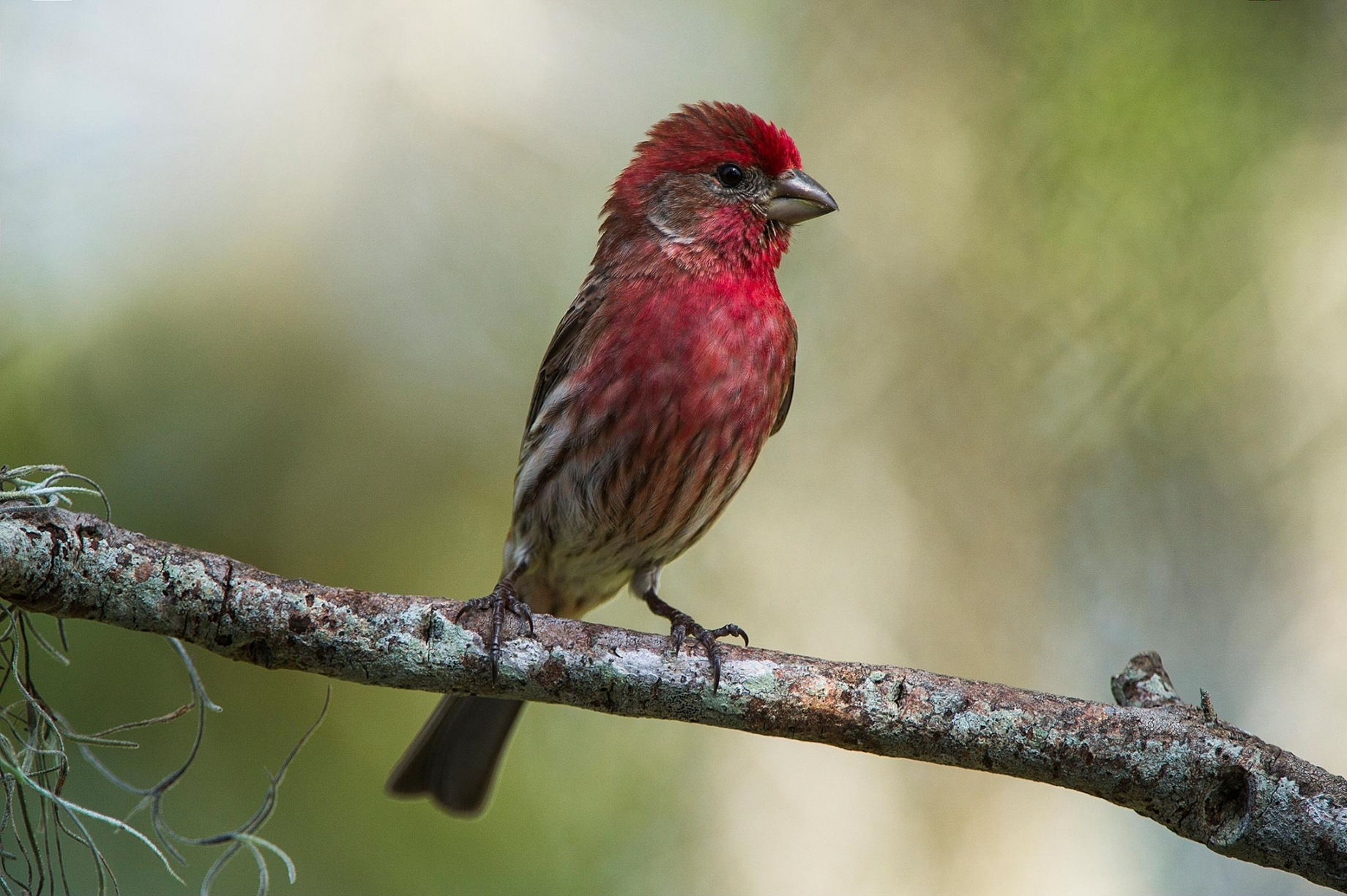
Birds such as the house finch are the natural host of
© Pete Oxford/Minden Pictures/Corbis
The peak period when ticks are seeking hosts starts earlier in the warmer, more southern, states than in northern states.43 Correspondingly, the onset of human Lyme disease cases occurs earlier as the growing degree days (a measurement of temperature thresholds that must be met for biological processes to occur) increases, yet, the timing of the end of the Lyme disease season does not appear to be determined by weather-related variables.23 Rather, the number of potential carriers (for example, deer, birds, and humans) likely influences the timing of the end of the Lyme disease season.
The effects of temperature and humidity or precipitation on the seasonal activity patterns of nymphal western blacklegged ticks is more certain than the impacts of these factors on the timing of Lyme disease case occurrence.35,36 Peak nymphal activity is generally reached earlier in hotter and drier areas, but lasts for shorter durations. Host-seeking activity ceases earlier in the season in cooler and more humid conditions. The density of nymphal western blacklegged ticks in north-coastal California consistently begins to decline when average daily maximum temperatures are between 70°F (21°C) and 73.5°F (23°C), and when average maximum daily relative humidity decreases below 83%–85%.35,36
Projected Impacts
Warmer winter and spring temperatures are projected to lead to earlier annual onset of Lyme disease cases in the eastern United States (see “Research Highlight” below) and in an earlier onset of nymphal host-seeking behavior.59 Limited research shows that the geographic distribution of blacklegged ticks is expected to expand to higher latitudes and elevations in the future and retract in the southern United States.60 Declines in subfreezing temperatures at higher latitudes may be responsible for improved survival of ticks. In many woodlands, ticks can find refuge from far-subzero winter air temperatures in the surface layers of the soil.61,62 However, a possibly important impact of climate change will be acceleration of the tick life cycles due to higher temperatures during the spring, summer, and autumn, which would increase the likelihood that ticks survive to reproduce.51,63 This prediction is consistent with recent observations of the spread of I. scapularis in Canada.48,64
To project accurately the changes in Lyme disease risk in humans based on climate variability, long-term data collection on tick vector abundance and human infection case counts are needed to better understand the relationships between changing climate conditions, tick vector abundance, and Lyme disease case occurrence.
State of the Science
Figure 5.5: Incidence of West Nile Neuroinvasive Disease by County in the United States
The majority (70% to 80%) of people infected with WNV do not show symptoms of the disease. Of those infected, 20% to 30% develop
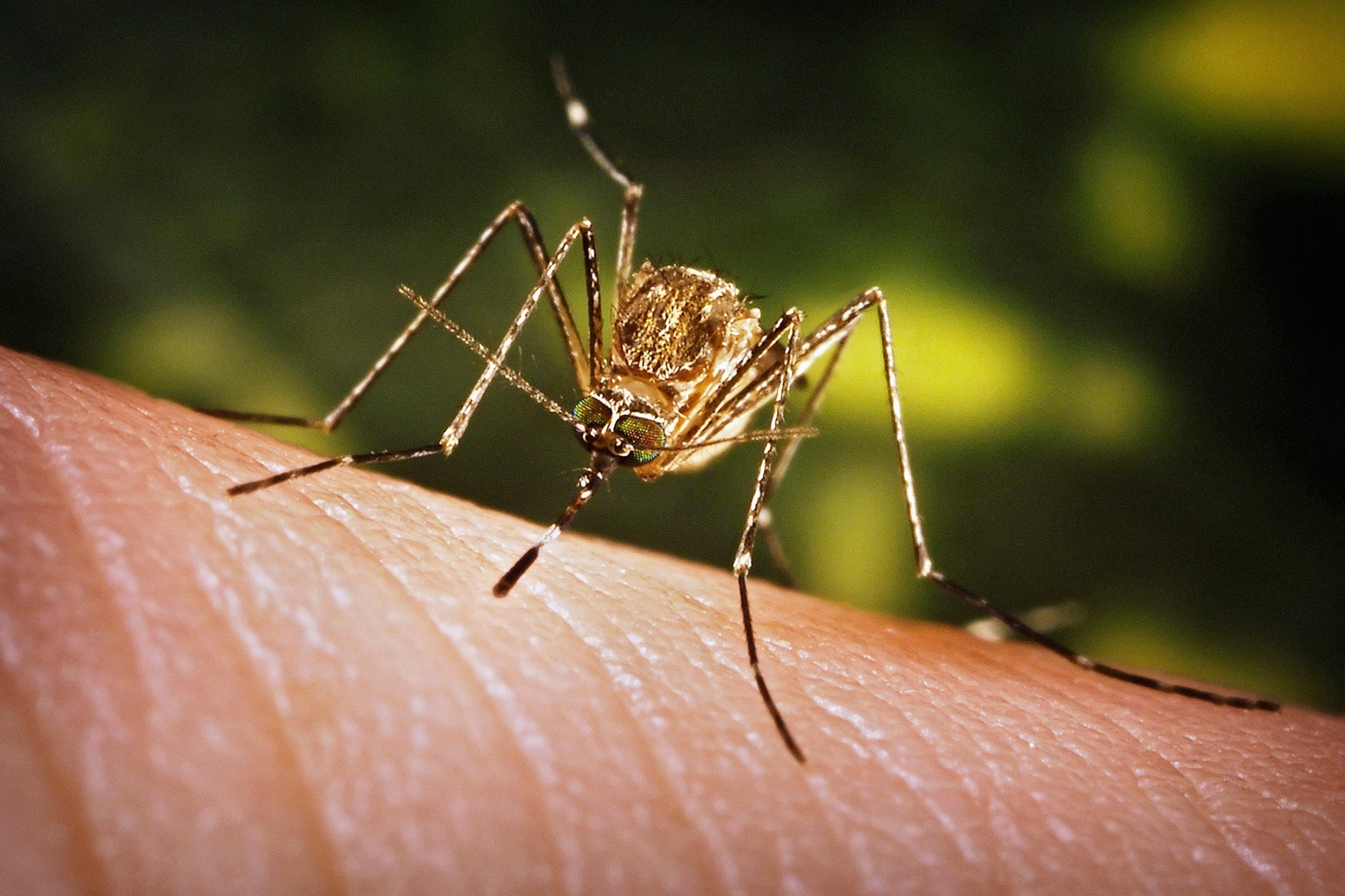
Humans can be infected from a bite of a mosquito that has previously bitten an infected bird.
© CDC/Science Faction/Corbis
West Nile virus is maintained in transmission cycles between birds (the natural hosts of the virus) and mosquitoes (Figure 5.6). The number of birds and mosquitoes infected with WNV increases as mosquitoes pass the virus from bird to bird starting in late winter or spring. Human infections can occur from a bite of a mosquito that has previously bitten an infected bird.81 Humans do not pass on the virus to biting mosquitoes because they do not have sufficient concentrations of the virus in their bloodstreams.82,83 In rare instances, WNV can be transmitted through blood transfusions or organ transplants.82,84 Peak transmission of WNV to humans in the United States typically occurs between June and September, coinciding with the summer season when mosquitoes are most active and temperatures are highest.85
Figure 5.6: Climate Impacts on West Nile Virus Transmission
Observed Impacts and Indicators
Mosquito vectors and bird hosts are required for WNV to persist, and the dynamics of both are strongly affected by climate in a number of ways. Geographical variation in average climate constrains the ranges of both vectors and hosts, while shorter-term
WNV has been detected in 65 mosquito species and more than 300 bird species in the United States,85 although only a relatively small number of these species contribute substantively to human infections. Three Culex (Cx.) mosquito species are the primary vectors of the virus in different regions of the continental United States, and differences in their preferred breeding habitats mean that
Impacts of Climate and
Climate, or the long-term average weather, is important for defining WNV’s transmission range limits because extreme conditions—too cold, hot, wet, or dry—can alter mosquito and bird habitat availability, increase
Climate change may influence mosquito survival rates through changes in season length, although mosquitoes are also able to adapt to changing conditions. For example, mosquitoes that transmit WNV are limited to latitudes and altitudes where winters are short enough for them to survive.88 However, newly emerged adult female mosquitoes have some ability to survive cold temperatures by entering a reproductive arrest called diapause as temperatures begin to cool and days grow shorter in late summer.89,90 These females will not seek a blood meal until temperatures begin to warm the following year. Even during diapause, very harsh winters may reduce mosquito populations, as temperatures near freezing have been shown to kill diapausing Cx. tarsalis.91
During the warmer parts of the year, Culex mosquitoes must have aquatic habitat available on a nearly continuous basis because their eggs hatch within a few days after they are laid and need moisture to remain viable. The breeding habitats of WNV vectors vary by species, ranging from fresh, sunlit water found in irrigated crops and wetlands preferred by Cx. tarsalis to stagnant, organically enriched water sources such as urban storm drains, unmaintained swimming pools, or backyard containers used by Cx. pipiens and Cx. quinquefasciatus92,93,94
WNV has become
Temperature is the most studied climate driver of the dynamics of WNV transmission. It is clear that warm temperatures accelerate virtually all of the biological processes that affect transmission: accelerating the mosquito life cycle,100,101,102,103,104 increasing the mosquito biting rates that determine the frequency of contact between mosquitoes and hosts,105,106 and increasing viral replication rates inside the mosquito that decrease the time needed for a blood-fed mosquito to be able to pass on the virus.107,108,109 These relationships between increasing temperatures and the biological processes that affect WNV transmission suggest a subsequent increase in risk of human disease.110,111,112,113 However, results from models have suggested that extreme high temperatures combined with decreased precipitation may decrease mosquito populations.114
Precipitation can create aquatic breeding sites for WNV vectors,115,116 and in some areas snowpack increases the amount of stored water available for urban or agricultural systems, which provide important habitat for WNV vectors,117,118 although effects depend on human water management decisions and vary spatially.101 Droughts have been associated with increased WNV activity, but the association between decreased precipitation and WNV depends on location and the particular sequence of
The impact of year-to-year changes in precipitation on mosquito populations varies among the regions of the United States and is affected by the typical climate of the area as well as other non-climate factors, such as
Impacts of Long-Term Climate Trends
The relatively short period of WNV’s transmission in the United States prevents direct observation of the impacts of long-term climate trends on WNV incidence. However, despite the short history of WNV in the United States, there are some lessons to be learned from other mosquito-borne diseases with longer histories in the United States.
Western equine encephalomyelitis virus (WEEV) and St. Louis encephalitis virus (SLEV) were first identified in the 1930s and have been circulating in the United States since that time. Like WNV, both viruses are transmitted primarily by Culex mosquitoes and are climate-sensitive. WEEV outbreaks were associated with wet springs followed by warm summers.118,126 Outbreaks of SLEV were associated with hot, dry periods when urban mosquito production increased due to stagnation of water in underground systems or when cycles of drought and wetting set up more complex transmission dynamics.127,128
Despite climatic warming that would be expected to favor increased WEEV and SLEV transmission, both viruses have had sharply diminished incidence during the past 30 to 40 years.129,130 Although the exact reason for this decline is unknown, it is likely a result of non-climate factors, such as changes in human behavior or undetected aspects of viral evolution. Several other mosquito-borne pathogens, such as chikungunya and dengue, have grown in importance as global health threats during recent decades; however, a link to climate change induced disease expansion in the United States has not yet been confirmed. These examples demonstrate the variable impact that climate change can have on different mosquito-borne diseases and help to explain why the direction of future trends in risk for WNV remain unclear.
Projected Impacts
Given WNV’s relatively short history in the United States, the described geographic variation in climate responses, and the complexity of transmission cycles, projecting the future distribution of WNV under climate change remains a challenge. Despite the growing body of work examining the connections between WNV and weather, climate-based seasonal forecasts of WNV outbreak risk are not yet available at a national scale. Forecasting the annual presence of WNV disease on the basis of climate and other ecological factors has been attempted for U.S. counties, with general agreement between modeled expectations and observed data, but more quantitative predictions of disease incidence or the risk for human
Longer-term projections of WNV under climate change scenarios are also rare. WNV is projected to increase in much of the northern and southeastern United States due to rising temperatures and declining precipitation, respectively, with the potential for decreased occurrence across the central United States.132 Future projections show that the season when mosquitoes are most abundant will begin earlier and end later, possibly resulting in fewer mosquitoes in mid-summer in southern locations where extreme heat is predicted to coincide with decreased summer precipitation.114
In addition to climate factors, multiple non-climate factors also influence human
Individual characteristics, such as age, gender, and immune function, may also affect vulnerability by influencing susceptibility to infection.21,80,140,143,144,145 Lyme disease is more frequently reported in children between 5 and 9 years of age and in adults between the ages of 55 and 59,21 and advanced age and being male contribute to a higher risk for severe WNV infections.79,144,145
The impacts of climate change on human vulnerability to vector-borne disease may be minimized by individual- or community-level
Climate factors are useful benchmarks to indicate seasonal risk and broad geographic changes in disease occurrence over decades. However, human vulnerability to vector-borne disease is more holistically evaluated by examining climate factors with non-climate factors (environmental or institutional context, social and behavioral context, and individual characteristics). Ultimately, a community’s capacity to adapt to both the climate and non-climate factors will affect population vulnerability to vector-borne disease.
Some vector-borne diseases may be introduced or become re-established in the United States by a variety of mechanisms. In conjunction with trade and travel,
In addition to the emerging issues identified above, and based on their review of the literature, the authors highlight the following areas for potential scientific research activity on vector-borne disease.
Numerous studies have identified associations between vector-borne diseases and
The risk for vector-borne diseases is highly variable geographically and over time. Monitoring responses of pathogens to
Future assessments can benefit from research activities that:
- evaluate how climatic variables, socioeconomic factors, and human behavior influence vector-borne disease occurrence and are expected to affect human adaptive capacity and the ability to respond to future disease threats;
- enhance long-term, systematic data collection on vector and pathogen distributions to detect changes over time. Such datasets must span a range of land-use types, including urban areas, and should be coupled with data on human disease;
- utilize mechanistic models that provide an evidence-based view of climate’s impacts on vector-borne diseases by explicitly accounting for the series of discrete but intertwined events that give rise to transmission. Models should be supported and validated by data specific to the disease system and include a realistic assessment of parameter
uncertainty and variability; - study the natural maintenance cycles of vector-borne pathogen evolution, emergence, and transmission as well as how climatic variables influence these cycles.
References
- Effect of forest fragmentation on Lyme disease risk. Conservation Biology, 17, 267-272. doi:10.1046/j.1523-1739.2003.01260.x | Detail , 2003:
- Climatic analysis of Lyme disease in the United States. Climate Research, 27, 177-187. doi:10.3354/cr027177 | Detail , 2004:
- Surveillance for Lyme disease--United States, 1992-2006. Morbidity and Mortality Weekly Report - Surveillance Summaries, 57, 1-9. PMID: 18830214 | Detail , 2008:
- Mosquitoes and West Nile virus along a river corridor from prairie to montane habitats in eastern Colorado. Journal of Vector Ecology, 34, 276-293. doi:10.1111/j.1948-7134.2009.00036.x | Detail , 2009:
- Resurgence of West Nile neurologic disease in the United States in 2012: What happened? What needs to be done? Antiviral Research, 99, 1-5. doi:10.1016/j.antiviral.2013.04.015 | Detail , 2013:
- Non-heat related impacts of climate change on working populations. Global Health Action, 3, 5640. doi:10.3402/gha.v3i0.5640 | Detail , 2010:
- Microclimate-dependent survival of unfed adult Ixodes scapularis (Acari: Ixodidae) in nature: Life cycle and study design implications. Journal of Medical Entomology, 33, 619-627. doi:10.1093/jmedent/33.4.619 | Detail , 1996:
- Does high biodiversity reduce the risk of Lyme disease invasion? Parasites & Vectors, 6, 195. doi:10.1186/1756-3305-6-195 | Detail , 2013:
- A focus of Lyme disease in Monmouth County, New Jersey. American Journal of Epidemiology, 120, 387-394. PMID: 6475916 | Detail , 1984:
- Key role of T cell defects in age-related vulnerability to West Nile virus. The Journal of Experimental Medicine, 206, 2735-2745. doi:10.1084/jem.20090222 | Detail , 2009:
- A climate-based model predicts the spatial distribution of the Lyme disease vector Ixodes scapularis in the United States. Environmental Health Perspectives, 111, 1152-1157. doi:10.1289/ehp.6052 | Detail , 2003:
- Effect of climate change on Lyme disease risk in North America. EcoHealth, 2, 38-46. doi:10.1007/s10393-004-0139-x | Detail , 2005:
- Assessing the roles of temperature, precipitation, and ENSO in dengue re-emergence on the Texas-Mexico border region. Salud Publica Mex, 50, 227-234. doi:10.1590/S0036-36342008000300006 | Detail , 2008:
- Overwintering survival of nymphal Ixodes scapularis (Acari: Ixodidae) under natural conditions. Journal of Medical Entomology, 49, 981-987. doi:10.1603/me12060 | Detail , 2012:
- West Nile virus infections projected from blood donor screening data, United States, 2003. Emerging Infectious Diseases, 12, 395-402. doi:10.3201/eid1203.051287 | Detail , 2006:
- Combined sewage overflows (CSO) are major urban breeding sites for Culex quinquefasciatus in Atlanta, Georgia. The American Journal of Tropical Medicine and Hygiene, 77, 478-484. URL | Detail , 2007:
- Neuroinvasive disease and West Nile virus infection, North Dakota, USA, 1999–2008. Emerging Infectious Diseases, 18, 684-686. doi:10.3201/eid1804.111313 | Detail , 2012:
- Notice to readers: Final 2004 reports of Notifiable Diseases. MMWR. Morbidity and Mortality Weekly Report, 54, 770-780. URL | Detail 2005:
- Notice to readers: Final 2005 Reports of Notifiable Diseases. MMWR. Morbidity and Mortality Weekly Report, 55, 880-881. URL | Detail 2006:
- Notice to readers: Final 2006 Reports of Nationally Notifiable Infectious Diseases. MMWR. Morbidity and Mortality Weekly Report, 56, 853-863. URL | Detail 2007:
- Notice to readers: Final 2007 Reports of Nationally Notifiable Infectious Diseases. MMWR. Morbidity and Mortality Weekly Report, 57, 901,903-913. URL | Detail 2008:
- Notice to readers: Final 2008 Reports of Nationally Notifiable Infectious Diseases. MMWR. Morbidity and Mortality Weekly Report, 58, 856-869. URL | Detail 2009:
- Notice to readers: Final 2009 Reports of Nationally Notifiable Infectious Diseases. MMWR. Morbidity and Mortality Weekly Report, 59, 1027-1039. URL | Detail 2010:
- Notice to readers: Final 2010 Reports of Nationally Notifiable Infectious Diseases. MMWR. Morbidity and Mortality Weekly Report, 60, 1088-1101. URL | Detail 2011:
- Notice to readers: Final 2011 Reports of Nationally Notifiable Infectious Diseases. MMWR. Morbidity and Mortality Weekly Report, 61, 624-637. URL | Detail 2012:
- Notice to readers: Final 2013 Reports of Nationally Notifiable Infectious Diseases. MMWR. Morbidity and Mortality Weekly Report, 63, 702-715. URL | Detail 2014:
- Surveillance Resources: ArboNET. Centers for Disease Control and Prevention, Arboviral Diseases Branch. URL | Detail cited 2014:
- Lyme Disease: Data and Statistics: Maps- Reported Cases of Lyme Disease – United States, 2001-2014. Centers for Disease Control and Prevention. URL | Detail cited 2015:
- Ticks: Life Cycle of Hard Ticks that Spread Disease. Centers for Disease Control and Prevention. URL | Detail cited 2015:
- Reported Cases of Lyme Disease by Year, United States, 1995-2013. Centers for Disease Control and Prevention. URL | Detail cited 2015:
- Lyme Disease: Preventing Tick Bites on People. Centers for Disease Control and Prevention. URL | Detail cited 2015:
- Notice to readers: Final 2012 Reports of Nationally Notifiable Infectious Diseases. Morbidity and Mortality Weekly Report, 62, 669-682. PMID: 24133698 | Detail 2013:
- Weather and land cover influences on mosquito populations in Sioux Falls, South Dakota. Journal of Medical Entomology, 48, 669-679. doi:10.1603/me10246 | Detail , 2011:
- Evidence implicating nymphal Ixodes pacificus (Acari, ixodidae) in the epidemiology of Lyme disease in California. The American Journal of Tropical Medicine and Hygiene, 53, 237-240. | Detail , 1995:
- Identification and Geographical Distribution of the Mosquitos of North America, North of Mexico. University Press of Florida, 383 pp. | Detail , 2005:
- Landscape, demographic, entomological, and climatic associations with human disease incidence of West Nile virus in the state of Iowa, USA. International Journal of Health Geographics, 7, 19. doi:10.1186/1476-072x-7-19 | Detail , 2008:
- Forum: Reported Distribution of Ixodes scapularis and Ixodes pacificus (Acari: Ixodidae) in the United States. Journal of Medical Entomology, 35, 629-638. doi:10.1093/jmedent/35.5.629 | Detail , 1998:
- The history of dengue outbreaks in the Americas. American Journal of Tropical Medicine and Hygiene, 87, 584-593. doi:10.4269/ajtmh.2012.11-0770 | Detail , 2012:
- Spatiotemporal Patterns of Host-Seeking Ixodes scapularis Nymphs (Acari: Ixodidae) in the United States. Journal of Medical Entomology, 43, 166-176. doi:10.1093/jmedent/43.2.166 | Detail , 2006:
- Field and climate-based model for predicting the density of host-seeking nymphal Ixodes scapularis, an important vector of tick-borne disease agents in the eastern United States. Global Ecology and Biogeography, 19, 504-514. doi:10.1111/j.1466-8238.2010.00526.x | Detail , 2010:
- Human Risk of Infection with Borrelia burgdorferi, the Lyme Disease Agent, in Eastern United States. The American Journal of Tropical Medicine and Hygiene, 86, 320-327. doi:10.4269/ajtmh.2012.11-0395 | Detail , 2012:
- Effects of larval rearing temperature on immature development and West Nile virus vector competence of Culex tarsalis. Parasites & Vectors, 5, 199. doi:10.1186/1756-3305-5-199 | Detail , 2012:
- Effect of environmental temperature on the ability of Culex pipiens (Diptera: Culicidae) to transmit West Nile virus. Journal of Medical Entomology, 39, 221-225. doi:10.1603/0022-2585-39.1.221 | Detail , 2002:
- Prevalence of West Nile virus in migratory birds during spring and fall migration. The American Journal of Tropical Medicine and Hygiene, 81, 1151-1158. doi:10.4269/ajtmh.2009.09-0106 | Detail , 2009:
- Irrigated agriculture is an important risk factor for West Nile virus disease in the hyperendemic Larimer-Boulder-Weld area of north central Colorado. Journal of Medical Entomology, 47, 939-951. doi:10.1093/jmedent/47.5.939 | Detail , 2010:
- Seasonal activity patterns of Ixodes pacificus nymphs in relation to climatic conditions. Medical and Veterinary Entomology, 16, 235-244. doi:10.1046/j.1365-2915.2002.00372.x | Detail , 2002:
- A spatially-explicit model of acarological risk of exposure to Borrelia burgdorferi-infected Ixodes pacificus nymphs in northwestern California based on woodland type, temperature, and water vapor. Ticks and Tick-Borne Diseases, 1, 35-43. doi:10.1016/j.ttbdis.2009.12.002 | Detail , 2010:
- Habitat-related variation in infestation of lizards and rodents with Ixodes ticks in dense woodlands in Mendocino County, California. Experimental and Applied Acarology, 33, 215-233. doi:10.1023/B:Appa.0000032954.71165.9e | Detail , 2004:
- Environmentally related variability in risk of exposure to Lyme disease spirochetes in northern California: Effect of climatic conditions and habitat type. Environmental Entomology, 32, 1010-1018. doi:10.1603/0046-225X-32.5.1010 | Detail , 2003:
- Diapause and related phenomena in Culex mosquitoes: Their relation to arbovirus disease ecology. Current Topics in Vector Research, 4, 1-28. doi:10.1007/978-1-4612-4712-8_1 | Detail , 1987:
- Increasing habitat suitability in the United States for the tick that transmits Lyme disease: A remote sensing approach. Environmental Health Perspectives, 110, 635-640. URL | Detail , 2002:
- Temporal relation between Ixodes scapularis abundance and risk for Lyme disease associated with erythema migrans. American Journal of Epidemiology, 149, 771-776. URL | Detail , 1999:
- Integrated assessment of behavioral and environmental risk factors for Lyme disease infection on Block Island, Rhode Island. PLoS ONE, 9, e84758. doi:10.1371/journal.pone.0084758 | Detail , 2014:
- Western equine encephalitis submergence: Lack of evidence for a decline in virus virulence. Virology, 380, 170-172. doi:10.1016/j.virol.2008.08.012 | Detail , 2008:
- Climate and vectorborne diseases. American Journal of Preventive Medicine, 35, 436-450. doi:10.1016/j.amepre.2008.08.030 | Detail , 2008:
- Gonotrophic cycle estimate for Culex quinquefasciatus in Mérida, Yucatán, México. Journal of the American Mosquito Control Association, 24, 344-348. doi:10.2987/5667.1 | Detail , 2008:
- Weather variability affects abundance of larval Culex (Diptera: Culicidae) in storm water catch basins in suburban Chicago. Journal of Medical Entomology, 49, 270-276. doi:10.1603/ME11073 | Detail , 2012:
- Modifiable Risk Factors for West Nile Virus Infection during an Outbreak--Arizona, 2010. The American Journal of Tropical Medicine and Hygiene, 86, 895-901. doi:10.4269/ajtmh.2012.11-0502 | Detail , 2012:
- Climate variability and change in the United States: Potential impacts on vector- and rodent-borne diseases. Environmental Health Perspectives, 109, 223-233. doi:10.2307/3435012 | Detail , 2001:
- Predicting the risk of Lyme disease: Habitat suitability for Ixodes scapularis in the north central United States. Emerging Infectious Diseases, 8, 289-297. doi:10.3201/eid0803.010166 | Detail , 2002:
- A continental risk assessment of West Nile virus under climate change. Global Change Biology, 20, 2417-2425. doi:10.1111/gcb.12534 | Detail , 2014:
- Effects of temperature on emergence and seasonality of West Nile virus in California. The American Journal of Tropical Medicine and Hygiene, 86, 884-894. doi:10.4269/ajtmh.2012.11-0342 | Detail , 2012:
- Epidemiology and transmission dynamics of West Nile virus disease. Emerging Infectious Diseases, 11, 1167-1173. doi:10.3201/eid1108.050289a | Detail , 2005:
- Microbial Threats to Health: Emergence, Detection, and Response. , Institute of Medicine. The National Academies Press, 398 pp. doi:10.17226/10636 | Detail 2003:
- Drought-induced amplification of local and regional West Nile virus infection rates in New Jersey. Journal of Medical Entomology, 50, 195-204. doi:10.1603/me12035 | Detail , 2013:
- Global trends in emerging infectious diseases. Nature, 451, 990-993. doi:10.1038/nature06536 | Detail , 2008:
- Geographical and environmental factors driving the increase in the Lyme disease vector Ixodes scapularis. Ecosphere, 3, art85. doi:10.1890/ES12-00134.1 | Detail , 2012:
- Globalization, land use, and the invasion of West Nile virus. Science, 334, 323-327. doi:10.1126/science.1201010 | Detail , 2011:
- Drivers, dynamics, and control of emerging vector-borne zoonotic diseases. The Lancet, 380, 1946-1955. doi:10.1016/s0140-6736(12)61151-9 | Detail , 2012:
- Temperature, viral genetics, and the transmission of West Nile virus by Culex pipiens mosquitoes. PLoS Pathogens, 4, e1000092. doi:10.1371/journal.ppat.1000092 | Detail , 2008:
- Host heterogeneity dominates West Nile virus transmission. Proceedings of the Royal Society B: Biological Sciences, 273, 2327-2333. doi:10.1098/rspb.2006.3575 | Detail , 2006:
- West Nile virus: Epidemiology and ecology in North America. The Flaviviruses: Detection, Diagnosis and Vaccine Development, , Elsevier Academic Press, 185-234. doi:10.1016/s0065-3527(03)61005-5 | Detail , 2003:
- Origin of the West Nile Virus responsible for an outbreak of encephalitis in the northeastern United States. Science, 286, 2333-2337. doi:10.1126/science.286.5448.2333 | Detail , 1999:
- Inter-annual associations between precipitation and human incidence of West Nile Virus in the United States. Vector-Borne and Zoonotic Diseases, 7, 337-343. doi:10.1089/vbz.2006.0590 | Detail , 2007:
- Diel activity of nymphal Dermacentor occidentalis and Ixodes pacificus (Acari: Ixodidae) in relation to meteorological factors and host activity periods. Journal of Medical Entomology, 32, 290-299. doi:10.1093/jmedent/32.3.290 | Detail , 1995:
- Lyme borreliosis: Relation of its causative agent to its vectors and hosts in North America and Europe. Annual Review of Entomology, 36, 587-609. doi:10.1146/annurev.en.36.010191.003103 | Detail , 1991:
- Predicting the speed of tick invasion: An empirical model of range expansion for the Lyme disease vector Ixodes scapularis in Canada. Journal of Applied Ecology, 49, 457-464. doi:10.1111/j.1365-2664.2012.02112.x | Detail , 2012:
- Accelerated phenology of blacklegged ticks under climate warming. Philosophical Transactions of the Royal Society B: Biological Sciences, 370. doi:10.1098/rstb.2013.0556 | Detail , 2015:
- Survival and development of Ixodes scapularis (Acari: Ixodidae) under various climatic conditions in Ontario, Canada. Journal of Medical Entomology, 32, 143-152. doi:10.1093/jmedent/32.2.143 | Detail , 1995:
- Impact of host community composition on Lyme disease risk. Ecology, 89, 2841-2849. doi:10.1890/07-1047.1 | Detail , 2008:
- Relation of temperature and humidity to winter survival of Culex pipiens and Culex tarsalis. Mosquito News, 21, 252-254. | Detail , 1961:
- Towards an early warning system for forecasting human west nile virus incidence. PLOS Currents: Disasters, 6. doi:10.1371/currents.outbreaks.f0b3978230599a56830ce30cb9ce0500 | Detail , 2014:
- Comparing the relative potential of rodents as reservoirs of the Lyme disease spirochete (Borrelia burgdorferi). American Journal of Epidemiology, 130, 143-150. PMID: 2787105 | Detail , 1989:
- Precipitation and the occurrence of Lyme disease in the northeastern United States. Vector-Borne and Zoonotic Diseases, 4, 143-148. doi:10.1089/1530366041210765 | Detail , 2004:
- Epidemiology of Lyme disease. Infectious Disease Clinics of North America, 29, 187-210. doi:10.1016/j.idc.2015.02.010 | Detail , 2015:
- Climate change influences on the annual onset of Lyme disease in the United States. Ticks and Tick-Borne Diseases, 6, 615-622. doi:10.1016/j.ttbdis.2015.05.005 | Detail , 2015:
- Meteorological influences on the seasonality of Lyme disease in the United States. The American Journal of Tropical Medicine and Hygiene, 90, 486-496. doi:10.4269/ajtmh.13-0180 | Detail , 2014:
- Regional and seasonal response of a West Nile virus vector to climate change. Proceedings of the National Academy of Sciences of the United States of America, 110, 15620-15625. doi:10.1073/pnas.1307135110 | Detail , 2013:
- Epidemic West Nile encephalitis, New York, 1999: Results of a household-based seroepidemiological survey. The Lancet, 358, 261-264. doi:10.1016/S0140-6736(01)05480-0 | Detail , 2001:
- Simulation of Management Strategies for the Blacklegged Tick (Acari: Ixodidae) and the Lyme Disease Spirochete, Borrelia burgdorferi. Journal of Medical Entomology, 34, 672-683. doi:10.1093/jmedent/34.6.672 | Detail , 1997:
- Off-host physiological ecology of ixodid ticks. Annual Review of Entomology, 36, 659-681. doi:10.1146/annurev.en.36.010191.003303 | Detail , 1991:
- Overwintering biology of Culex (Diptera: Culicidae) mosquitoes in the Sacramento Valley of California. Journal of Medical Entomology, 50, 773-790. doi:10.1603/me12280 | Detail , 2013:
- Current practices and evaluation of screening solid organ donors for West Nile virus. Transplant Infectious Disease, 14, 268-277. doi:10.1111/j.1399-3062.2012.00743.x | Detail , 2012:
- Recommendations for Protecting Outdoor Workers from West Nile Virus Exposure. 16 pp., Department of Health and Human Services, Centers for Disease Control and Prevention, National Institute for Occupational Safety and Health. URL | Detail 2005:
- Climate change and the potential for range expansion of the Lyme disease vector Ixodes scapularis in Canada. International Journal for Parasitology, 36, 63-70. doi:10.1016/j.ijpara.2005.08.016 | Detail , 2006:
- Risk maps for range expansion of the Lyme disease vector, Ixodes scapularis, in Canada now and with climate change. International Journal of Health Geographics, 7, 24. doi:10.1186/1476-072X-7-24 | Detail , 2008:
- Active and passive surveillance and phylogenetic analysis of Borrelia burgdorferi elucidate the process of Lyme disease risk emergence in Canada. Environmental Health Perspectives, 118, 909-914. doi:10.1289/ehp.0901766 | Detail , 2010:
- Environmental risk from Lyme disease in central and eastern Canada: A summary of recent surveillance information. Canada Communicable Disease Report, 40, 74-82. URL | Detail , 2014:
- Estimated effects of projected climate change on the basic reproductive number of the Lyme disease vector Ixodes scapularis. Environmental Health Perspectives, 122, 631-638. doi:10.1289/ehp.1307799 | Detail , 2014:
- Climate, deer, rodents, and acorns as determinants of variation in Lyme-disease risk. Plos Biology, 4, e145. doi:10.1371/Journal.Pbio.0040145 | Detail , 2006:
- Migrating birds as dispersal vehicles for West Nile virus. EcoHealth, 3, 79-85. doi:10.1007/s10393-006-0025-9 | Detail , 2006:
- Chikungunya. Pan American Health Organization. URL | Detail cited 2014:
- Climate, environmental and socio-economic change: Weighing up the balance in vector-borne disease transmission. Philosophical Transactions of the Royal Society B: Biological Sciences, 370. doi:10.1098/rstb.2013.0551 | Detail , 2015:
- Ecological and evolutionary responses to recent climate change. Annual Review of Ecology, Evolution, and Systematics, 37, 637-669. doi:10.1146/annurev.ecolsys.37.091305.110100 | Detail , 2006:
- Transmission of West Nile virus through blood transfusion in the United States in 2002. New England Journal of Medicine, 349, 1236-1245. doi:10.1056/NEJMoa030969 | Detail , 2003:
- Climatic and landscape correlates for potential West Nile virus mosquito vectors in the Seattle region. Journal of Vector Ecology, 32, 22-28. doi:10.3376/1081-1710(2007)32[22:CALCFP]2.0.CO;2 | Detail , 2007:
- Geographic variation in the relationship between human Lyme disease incidence and density of infected host-seeking Ixodes scapularis nymphs in the eastern United States. The American Journal of Tropical Medicine and Hygiene, 86, 1062-1071. doi:10.4269/ajtmh.2012.11-0630 | Detail , 2012:
- West Nile virus: Review of the literature. JAMA: The Journal of the American Medical Association, 310, 308-315. doi:10.1001/jama.2013.8042 | Detail , 2013:
- Westward ho?--The spread of West Nile virus. New England Journal of Medicine, 351, 2257-2259. doi:10.1056/NEJMp048261 | Detail , 2004:
- Estimated cumulative incidence of West Nile virus infection in US adults, 1999-2010. Epidemiology & Infection, 141, 591-595. doi:10.1017/S0950268812001070 | Detail , 2013:
- Transmission of Lyme disease spirochetes (Borrelia-Burgdorferi). Experimental and Applied Acarology, 7, 71-80. doi:10.1007/Bf01200454 | Detail , 1989:
- Dengue outbreak in Key West, Florida, USA, 2009. Emerging Infectious Diseases, 18, 135-137. doi:10.3201/eid1801.110130 | Detail , 2012:
- Epidemic dengue and dengue hemorrhagic fever at the Texas–Mexico border: Results of a household-based seroepidemiologic survey, December 2005. The American Journal of Tropical Medicine and Hygiene, 78, 364-369. URL | Detail , 2008:
- Epidemiology and Control of Mosquito-Borne Arboviruses in California, 1943-1987. California Mosquito and Vector Control Association, 508 pp. | Detail , 1990:
- A systematic review of mathematical models of mosquito-borne pathogen transmission: 1970-2010. Journal of the Royal Society Interface, 10. doi:10.1098/rsif.2012.0921 | Detail , 2013:
- Effect of temperature on Culex tarsalis (Diptera: Culicidae) from the Coachella and San Joaquin Valleys of California. Journal of Medical Entomology, 32, 636-645. doi:10.1093/jmedent/32.5.636 | Detail , 1995:
- Impact of climate variation on mosquito abundance in California. Journal of Vector Ecology, 33, 89-98. doi:10.3376/1081-1710(2008)33[89:iocvom]2.0.co;2 | Detail , 2008:
- Ecological observations on the 1989 outbreak of St. Louis encephalitis virus in the southern San Joaquin Valley of California. Journal of Medical Entomology, 29, 472-482. doi:10.1093/jmedent/29.3.472 | Detail , 1992:
- Limited interdecadal variation in mosquito (Diptera: Culicidae) and avian host competence for Western equine encephalomyelitis virus (Togaviridae: Alphavirus). The American Journal of Tropical Medicine and Hygiene, 78, 681-686. URL | Detail , 2008:
- Avian host and mosquito (Diptera: Culicidae) vector competence determine the efficiency of West Nile and St. Louis encephalitis virus transmission. Journal of Medical Entomology, 42, 367-375. doi:10.1603/0022-2585(2005)042[0367:ahamdc]2.0.co;2 | Detail , 2005:
- Effects of temperature on the transmission of West Nile virus by Culex tarsalis (Diptera: Culicidae). Journal of Medical Entomology, 43, 309-317. doi:10.1093/jmedent/43.2.309 | Detail , 2006:
- Texas lifestyle limits transmission of dengue virus. Emerging Infectious Diseases, 9, 86-89. doi:10.3201/eid0901.020220 | Detail , 2003:
- Transovarial transmission of Borrelia spirochetes by Ixodes scapularis: A summary of the literature and recent observations. Ticks and Tick-Borne Diseases, 4, 46-51. doi:10.1016/j.ttbdis.2012.06.008 | Detail , 2013:
- Search strategy has influenced the discovery rate of human viruses. Proceedings of the National Academy of Sciences of the United States of America, 110, 13961-13964. doi:10.1073/pnas.1307243110 | Detail , 2013:
- Temperature-dependent development and survival rates of Culex quinquefasciatus and Aedes aegypti (Diptera: Culicidae). Journal of Medical Entomology, 27, 892-898. doi:10.1093/jmedent/27.5.892 | Detail , 1990:
- Local impact of temperature and precipitation on West Nile virus infection in Culex species mosquitoes in northeast Illinois, USA. Parasites & Vectors, 3, Article 19. doi:10.1186/1756-3305-3-19 | Detail , 2010:
- Meteorologically Mediated Diurnal Questing of Ixodes scapularis and Amblyomma americanum (Acari: Ixodidae) Nymphs. Journal of Medical Entomology, 40, 395-402. doi:10.1603/0022-2585-40.4.395 | Detail , 2003:
- Precipitation and Temperature as Predictors of the Local Abundance of Ixodes scapularis (Acari: Ixodidae) Nymphs. Journal of Medical Entomology, 46, 1025-1029. doi:10.1603/033.046.0508 | Detail , 2009:
- Lyme disease in outdoor workers: Risk factors, preventive measures, and tick removal methods. American Journal of Epidemiology, 131, 877-885. | Detail , 1990:
- Longitudinal study of Borrelia burgdorferi infection in New Jersey outdoor workers, 1988-1991. American Journal of Epidemiology, 139, 504-512. | Detail , 1994:
- The spatial-temporal distribution of drought, wetting, and human cases of St. Louis encephalitis in southcentral Florida. The American Journal of Tropical Medicine and Hygiene, 71, 251-261. URL | Detail , 2004:
- Drought-induced amplification and epidemic transmission of West Nile virus in southern Florida. Journal of Medical Entomology, 42, 134-141. doi:10.1093/jmedent/42.2.134 | Detail , 2005:
- Hydrologic conditions describe West Nile virus risk in Colorado. International Journal of Environmental Research and Public Health, 7, 494-508. doi:10.3390/ijerph7020494 | Detail , 2010:
- Meteorological and hydrological influences on the spatial and temporal prevalence of West Nile virus in Culex mosquitos, Suffolk County, New York. Journal of Medical Entomology, 48, 867-875. doi:10.1603/ME10269 | Detail , 2011:
- Infectious disease in a warming world: How weather influenced West Nile virus in the United States (2001–2005). Environmental Health Perspectives, 117, 1049-1052. doi:10.1289/ehp.0800487 | Detail , 2009:
- Survival of immature Ixodes scapularis (Acari: Ixodidae) at different relative humidities. Journal of Medical Entomology, 31, 310-314. doi:10.1093/jmedent/31.2.310 | Detail , 1994:
- Beyond Lyme: Aetiology of tick-borne human diseases with emphasis on the south-eastern United States. Zoonoses and Public Health, 59, 48-64. doi:10.1111/j.1863-2378.2012.01475.x | Detail , 2012:
- Effects of climate on variability in Lyme disease incidence in the northeastern United States. American Journal of Epidemiology, 157, 531-538. doi:10.1093/aje/kwg014 | Detail , 2003:
- Effects of landscape fragmentation and climate on Lyme disease incidence in the northeastern United States. Ecohealth, 10, 394-404. doi:10.1007/s10393-013-0890-y | Detail , 2013:
- Effect of latitude on the rate of change in incidence of Lyme disease in the United States. CMAJ Open, 1, E43-E47. doi:10.9778/cmajo.20120002 | Detail , 2013:
- Dengue Fever (Locally Acquired) Human 2013. Cumulative data as of May 7, 2014. United States Geological Survey. URL | Detail cited 2014:
- Air temperature and relative humidity effects on behavioral activity of blacklegged tick (Acari: Ixodidae) nymphs in New Jersey. Journal of Medical Entomology, 35, 1025-1028. doi:10.1093/jmedent/35.6.1025 | Detail , 1998:
- Variation in life table characteristics among three geographic strains of Culex pipiens quinquefasciatus. Journal of Medical Entomology, 11, 541-550. | Detail , 1974:
- Relationships among weather, mosquito abundance, and encephalitis virus activity in California: Kern County 1990-98. Journal of the American Mosquito Control Association, 16, 22-27. | Detail , 2000:
- Clinical findings of West Nile virus infection in hospitalized patients, New York and New Jersey, 2000. Emerging Infectious Diseases, 7, 654-658. | Detail , 2001:
- Dengue and Severe Dengue. Fact Sheet No. 117. World Health Organization. URL | Detail cited 2015:
- Regional variation of climatic influences on West Nile virus outbreaks in the United States. The American Journal of Tropical Medicine and Hygiene, 91, 677-684. doi:10.4269/ajtmh.14-0239 | Detail , 2014:
- Predictive spatial models for risk of West Nile virus exposure in eastern and western Colorado. The American Journal of Tropical Medicine and Hygiene, 79, 581-590. URL | Detail , 2008:
- Duration and regulation of the developmental cycle of Ixodes dammini (Acari: Ixodidae). Journal of Medical Entomology, 27, 196-201. doi:10.1093/jmedent/27.2.196 | Detail , 1990:
- West Nile fever characteristics among viremic persons identified through blood donor screening. The Journal of Infectious Diseases, 202, 1354-1361. doi:10.1086/656602 | Detail , 2010: